Nature of Dark Matter - by Ricky Leon Murphy:
The Source of Heated Debates
How we know dark matter
exists
Importance of Computer
Simulation
Initial Theories - MACHO's
Hot Dark Matter
Warm Dark Matter
Cold Dark Matter
Testing Cold Dark
Matter
Radio Jets
DAMA Experiment
Self Interacting Dark Matter
Tachyons
Summary
Image Credits
Web Sites
References
Back to Cosmology
90% of all the material
in the Universe is of material we cannot see. While this seems to be an
almost embarrassing situation for Astronomers, super-computer
simulations, improved methods of observation and particle physics are
providing more plausible models of dark matter. While the results so far
have only ruled out previous contenders – like Modified Newtonian
Dynamics (MOND) and Massive Compact Objects (MACHO’s) – there are still
unanswered questions. Hot, Warm, and Cold Dark Matter – composed of
small, weakly interactive particles – are the more recent models with
the Cold Dark Matter (CDM) model the current reigning champ. In
addition, observational data, new computer simulations, and the
constraint of the age of the Universe have eliminated the hot and warm
dark matter models. Although the CDM model is the current favorite, it
is not without its share of problems. Self-Interacting dark matter and
Tachyonic dark matter are geared towards supporting the difficulties of
the CDM theory, but are still theoretical. The hope of repeated direct
detection of the CDM particles will soon be a reality, so we may one day
finally have direct, observational evidence of dark matter.
Back to Top
| Back to
Cosmology
The source of heated
debates
It is really hard to
imagine that any topic in science can be the source of targeted and
contemptuous debates. With regards to dark matter, the camps have
clearly demarcated battle lines, and the scouts are on the lookout. Just
how heated can these debates be? One of the many ideas of dark matter is
the use of modified Newtonian dynamics, or MOND. It is suggested by MOND
that dark matter has been miscalculated based on the acceleration curves
of galaxies (Kaplinghat and Turner, 2002). In addition, it is the
believed that the CDM computer simulations confirm the MOND ideas. While
MOND is the primary source of the dark matter theory, it was not the
intention of the author that the particles of the CDM theory be
eliminated (Kaplinghat and Turner, 2002). In response to this, a paper
was submitted a few months after directly attacking the Kaplinghat and
Turner 2002 paper (KT). It is argued that the KT paper only addresses
the dark matter constituents residing in the halo of single galaxies
only, and states that their mechanics in describing the galactic
acceleration curves are incorrect (Milgrom, 2002). This paper uses words
like “crude” and “phenomenologically wrong” and basically misreads the
KT paper in assuming the authors completely disregarded the constituents
of dark matter altogether.
While our observations do
not agree with MOND, it is clear that such debates exist and the ideas
and theories of dark matter have the ability to divide the world of
Astronomy and Astrophysics.
Back to Top
| Back to
Cosmology
How do we know Dark
Matter exists?
Our understanding of
Newtonian and Keplerian mechanics is quite good. After all, the planet
Neptune was predicted to be in its exact spot when finally viewed with a
telescope using this same math. We can map the orbits of planets, and
map the rotation curves of galaxies; however, the galactic rotation
curves turned out to be problematic. In Keplerian mechanics, as an
orbiting object is father from its point of rotation, the speed of
rotation is decreased (figure 1).
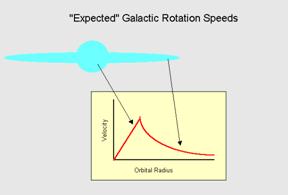 |
Figure 1:
Using Keplerian mathematics, this is the expected rotation curve
of a galaxy. It turns out that every observed galaxy does not
have this expected curve. |
Instead of the standard
curve, our actual measurements of galactic rotation is a bit different,
indicating that something massive must exist beyond the visible edge of
a galaxy (figure 2).
Figure 2: An
actual rotation curve of our own galaxy – The Milky Way. This
type of curve has been seen in every galaxy we have studied,
indicating the same phenomenon is present in all galaxies. The
phenomenon is dark matter. |
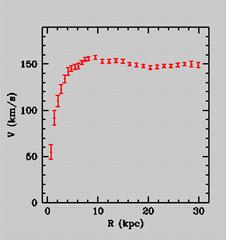 |
In addition to these
rotation curves, another indicator that dark matter exists is the
presence of lensing by individual galaxies and galaxy clusters.
Einstein’s theory of Relativity tells us that mass act upon everything,
including light. The easiest explanation is that all things in space
move in straight line, but their paths are altered by distortions in
space-time. An example: the orbit of Mercury about the Sun. Imagine
space-time as a rubber sheet, and our Sun is placed in the center. Since
the Sun is much more massive than anything else in our Solar System, a
very large depression is made. The path of Mercury is in reality
straight, but because it is close to the Sun, and stuck within the
depression made by the Sun, it is traveling in a straight line within
this curve of space-time (figure 3).
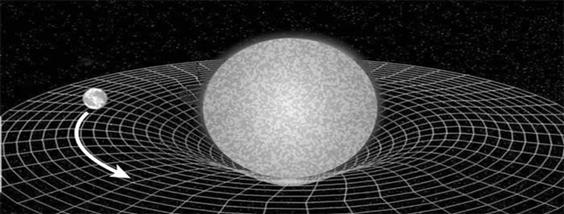 |
Figure 3: The
Sun creates a depression in Space-Time, and the orbiting planet
is caught in the curve. |
Figures 4, 5, 6, 7 and 8
show just how mass can affect the path of light. The gravity lens is
strongest proof we have that dark matter does exist.
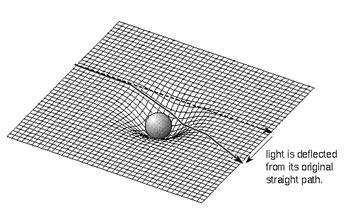 |
Figure 4: As
light travels near a depression of space-time, the path of light
is altered. |
Figure 5: As
seen from Earth, the position of a star near the Sun appears to
shift in position. |
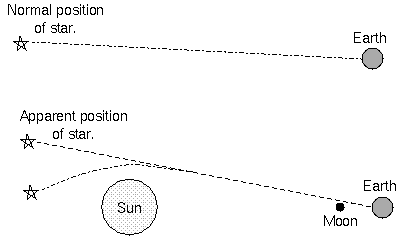 |
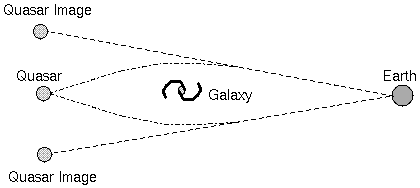 |
Figure 6: A
massive object, like a galaxy, can act like a lens. The result
is the diffraction of light around the massive object that
results in what is called the Einstein cross. |
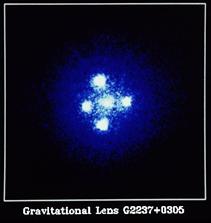 |
Figure 7: A
photographic example of an Einstein cross. This image
corresponds to figure 7. The object in the center is a galaxy
that lies between us and the quasar. The quasar is beyond the
galaxy, but the mass of the galaxy acts as a lens. The four
objects that make up the cross are actually the single quasar
that lies beyond. |
 |
Figure 8: The
greatest example of a gravity lens. Galaxy cluster Abell 2218
has enough mass that multiple distant objects appear as arcs in
the image. The visible mass alone cannot provide the required
mass to create such distortions in space-time. The only solution
is the introduction of dark matter. |
The Tully-Fisher
correlation (the correlation of a galaxy’s luminosity versus its
rotational velocity) proves the existence of dark matter (Silk, 1999).
With the affects of the gravity lens on the scale of galaxy clusters
indicate that dark matter exists around individual galaxies, and in
between galaxies that are members of a cluster or a group.
Back to Top
| Back to
Cosmology
The Importance of
Computer Simulations
It is widely accepted
that our Universe came to be by way of the Big Bang. Why the Big Bang
occurred is an answer that is pretty tough to solve, but we know there
was a big bang by studying the results of the Wilkinson Microwave
Anisotropy Probe (WMAP). The entire Universe was the target of this
probe, mapping the background microwave radiation – the leftovers from
the Big Bang. Not only does this background radiation demonstrate that
such a big bang occurred, the speed of the galaxies increase with
increasing distance from us (also called the Hubble Law). With the age
of the Universe at 13.7 billion years, we now have one variable solved
when we run computer simulations of our Universe. It is also generally
accepted by Astronomers that the hydrogen atoms did not start to form
until 300,000 years after the Big Bang – the temperature of the Universe
was too hot prior to this (again, as indicated by the background
radiation). We are sure dark matter exists in the Universe today, so it
follows that dark matter must have existed during the early formation of
the Universe. One of the particles believed to be holdovers from the Big
Bang are neutrinos. Because we know this particle exists, and is weakly
interactive with matter, the neutrino is the ingredient of the Hot Dark
Matter model – which we now know to be incorrect.
It is very difficult to
view the Universe in its infant stages of matter formation, so we must
rely on computer simulations. Computer programs are written to create a
single particle conforming to a series of parameters – like mass, size,
speed of movement, and so on. Multiply this by about a million (or so),
and then write another program on how each particle responds to each
other, again multiplied by about a million (or so). The end result is a
program that cannot possibly be run on a stand-alone PC. Dozens of
computers are tied together and controlled by a cluster manager server
that uses all of the processors to run this program. This is called a
super-computer. While it is beyond the scope of this project to
demonstrate the intricacies of using a super-computer to create
simulations of dark matter, we will only focus on the results. What we
have learned is that dark matter shaped our Universe by creating strings
of material that attracted matter gravitationally to create structures
like star clusters, galaxies, and galaxy clusters. An example of a small
section of a simulated Universe is shown in figure 9.
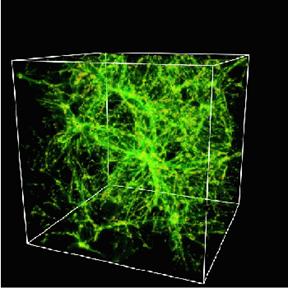 |
Figure 9: The
shows an end result of a computer simulated Universe. All of the
strings indicate the distribution of all known galaxies and
galaxy clusters. This type of model is often called an N-Body
Hydrodynamic model (Silk, 1999). |
Back to Top
| Back to
Cosmology
Initial Theories of
Dark Matter
Dark Matter – MACHO’s
not macho enough:
One of the previous
favorites of dark matter is the massive compact objects, or MACHO’s.
These baryonic
objects are: black holes, neutron stars, white dwarfs, red dwarfs, and
brown dwarfs. The stellar remnants – black holes, neutron stars and
white dwarfs – are very small and contain significant amounts of mass in
a fairly compact size. Red and brown dwarfs are not as massive. Brown
dwarfs are stars that have not initiated hydrogen fusion. All of these
objects would occupy the galactic halo – an imaginary bubble that
surrounds an entire galaxy that contains very old stars, globular
clusters and dark matter. An immediate problem regarding the theory of
MACHO’s is the lack of explanation to the constituents of dark matter
that lies in between galaxies within clusters. In addition, observations
by the Hubble Space telescope have ruled out any significant
contributions of dark matter by red and brown dwarfs (Flynn, Gould and
Bahcall, 1996). The Hubble Space Telescope data showed that white dwarfs
could provide almost 2% of the mass of the galactic halo, but further
simulations and re-evaluations of orbital kinematics place the white
dwarf contributions to only 1% (Gibson and Flynn, 2001). Further
simulations of the white dwarf contribution show that they do not
provide mass to the halo, but are a part of the galactic thick disk
(Reid, Sahu and Hawley, 2001).
What is most interesting
about the thick disk is its role in helping to confirm the existence of
Cold Dark Matter. Using computer simulations, it has been shown that the
thick disk of our galaxy was formed with the assistance of CDM, and that
the thick disk formed first (Brook, Kawata, Gibson, and Freeman, 2004).
This fits the paradigm of the CDM simulations that violent merging and
abrupt star formation helped to form the thick disk.
Back to Top
| Back to
Cosmology
Hot Dark Matter – what
happened?
The HDM model was favored
initially because it contains particles we know to exist – neutrinos.
The problem with the HDM model is twofold: firstly, when the theory was
put together, neutrinos were though to have either zero mass, or very
little. The recent observations with the Sudbury Neutrino Observatory
indicates that neutrinos oscillate – indicating mass, thereby solving
the infamous Solar Neutrino Problem. Secondly, the model of the HDM
Universe was based on relativistic, fast traveling, zero mass particles
(neutrinos) that resulted in creating only large scale structures – like
galaxy clusters – but failed to develop the smaller individual galaxies.
Even factoring in neutrino mass, the HDM model was unsuccessful in
creating individual galaxies. The answer to the HDM model: Cold Dark
Matter and Warm Dark Matter.
Figures 10 and 11 shows a
side by side comparison of the CDM and HDM models; notice the HDM image
with large scale structures only as compared with the CDM model which
shows a more pronounced structure of smaller density regions. The CDM
model is more consistent with what we observe.
So why exactly has the
HDM been abandoned? There are four reasons (Bode, Ostriker, and Turok):
- The particles speed
is too high
- High particle speed
eliminated perturbations required for large structures
- Galaxies formed
through fragmentation
- Predicted redshifts
far lower than observed redshifts
These four reasons are
the results of computer simulations.
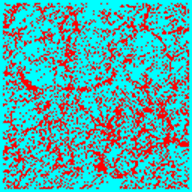 |
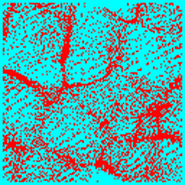 |
Figure 10:
The end result of a CDM simulation – more detail. |
Figure 11:
The end result of a HDM simulation – large structures only. |
Back to Top
| Back to
Cosmology
Warm Dark Matter
Cold Dark Matter was
modeled prior to the Warm Dark Matter model, but we will cover briefly
the WDM model only because it has since been abandoned in favor of the
CDM model due to recent observational evidence. Both WDM and CDM models
demonstrate a Universe that is similar to what we observe. However, the
problem with the CDM model is too many dwarf galaxies are present in the
halos of normal galaxies in computer simulations. Initially, the CDM and
the HDM models were compared, and the WDM model was introduced as the
middle ground between the two competing models (Silk, 1999). There are
really two main problems with the CDM Theory (Bode, Ostriker and Turok,
2001):
- The prediction of
numerous low mass galactic halo’s
- Prediction of
galactic halo’s with concentrated cores
Both of these issues are
present when running N-body simulations. It was believed the WDM model
would solve these issues simply by warming and smoothing out the
particles that constitute CDM. There are seven points of enhancement
noticed when the CDM was replaced with the WDM theory as modeled by
computer simulation (Bode, Ostiker and Turok, 2001):
- Smoothing of halo
cores and lowering core density
- Lower density of low
mass halos
- Reduced number of
low mass halos
- Suppressed number of
low mass halos within high mass halos
- The voids between
structure remain empty (unlike CDM with empty halos filling the
void)
- late-formation of
low mass halos
- suppressed halo
formation at high redshifts
While these seven points
of correction are compelling, Metcalf (2002) points out that there is no
evidence one way or another that empty halo objects within the voids of
space do not exists – they very well could exist but their nature
prevents direct observation.
So what happened to the
WDM model? The results from the WMAP probe placed some serious
constraints on this model. The re-ionization period of the Universe is
when hydrogen was cool enough to interact with each other. Based on the
computer models with the new WMAP data included, the WDM model must
rapidly increase its ionization factor (which it cannot by design) to
match the new constraint (Yoshida et al., 2003).
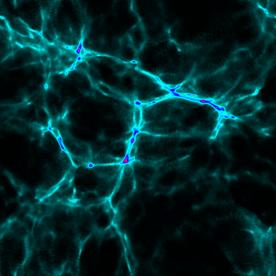 |
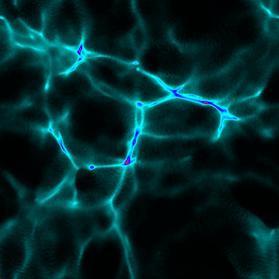 |
Figure 12: A
CDM sample simulation (on a better computer that figure 11),
showing the similar structure of our observed Universe. |
Figure 13: A
WDM sample simulation showing an almost identical overall
structure, but arrears to be more smooth as well. |
Figures 12 and 13 show a
single frame example of CDM versus WDM. The WDM simulation looks visibly
smooth but we now know the WDM model does not work.
Back to Top
| Back to
Cosmology
Accepted and current
theories of Dark Matter – CDM
The success of the
Cold Dark Matter model:
Neutrinos were
relativistic since the formation of the galaxies making them hot (Gondolo,
2004). Since the neutrino is the only weak interactive particle that we
know to exist, and because they are considered hot, they are a poor
choice for the ingredient of dark matter. The CDM model relies on
undiscovered weakly interactive particles that make up dark matter. The
problem is the model with the parameters fitting these particles
demonstrates a fairly successful representation of our Universe; but
what are these particles? The primary ingredient of CDM is non-baryonic
WIMP’s (Weakly Interacting Massive Particles). A baryon is simply the
smallest parts of matter as we know them: protons, neutrons, and
electrons. Leptons are electrons and neutrinos, and are also called
non-baryonic. Since neutrinos are ruled out as a constituent of CDM,
other non-baryonic particles are candidates. Since we do not know if
they really exist, we cannot call them leptons. With names like
neutralinos, axions, WIMPZILLA’s as candidates (Gondolo, 2004), it is
hard to imagine that these really do exist. Because the CDM model has
endured computer simulation models and observational data – with only
“minor” issues – the task is to attempt to determine why this model has
trouble with galactic halos being either empty or too compact – and of
course attempt to detect these unusually named particles.
One method that has been
suggested is to redo the entire simulation process. In a paper by Ma and
Bertschinger (2004), it is suggested that the N-body simulations is
flawed and will always lead to the “cuspy halo” and “dwarf satellite
problem.” By creating a model based on cosmological theory and
perturbation theory, the “phase-space distribution of dark matter
particles in galaxy halos” will be formed without the two major problems
resulting from the standard N-body simulations.
Back to Top
| Back to
Cosmology
Observational testing
of Cold Dark Matter:
We have seen that
observational data from the WMAP probe placed some death blows to the
WDM model, but what bout the CDM model? Currently there are three
methods to detect CDM:
- Examination of Radio
Jets
- DAMA Experiment
- WIMP detection using
CaWO4 cryogenic detectors
Back to Top
| Back to
Cosmology
Radio Jets:
In a paper by Metcalf
(2002), long term study of two radio jets from distant QSO’s
(Quasi-Stellar Objects) using the VLBI
array and examination of the radio jets show the images of the jets are
slightly bent. This is significant since the bending of the radio jets
are not consistent, indicating clumps of dark matter within the halos of
the early galaxies. This is also significant because this rules out the
WDM model since this model attempts to smooth halo formation. While this
may seem as just another confirmation of the CDM model, this is perhaps
one of the few direct observations of dark matter because its affects
can be measured directly over time.
Back to Top
| Back to
Cosmology
DAMA Experiment (http://www.lngs.infn.it/lngs/htexts/dama/welcome.html):
A remarkable experiment
called DAMA (DArk MAtter) has been using three styles of detectors in an
attempt to discover WIMP’s (in operation from the years 1997 to 2003).
This experiment looks exactly like the experiments to detect and study
neutrinos (figure 15), but the DAMA is looking for a specific reaction,
seen in figure 14. Specifically, the energy as the result of an
interaction with a particular element will be at a particular angle.
 |
Figure 14:
According to the DAMA website, WIMP’s are traveling within our
galaxy halo at 232 km/s. By measuring the affect of a WIMP (W)
on some other element (Mn), we can rule out other particles
(like neutrinos) that would also interact with the same
particle. |
The DAMA experiment was a
three phase process, with two R&D setups and one actual experiment based
on the results of the R&D. The idea behind the experiment is simple: As
the galaxy rotates (at 232 km/s) we are sweeping through the residual
CDM material in the halo. By studying the reactions of these particles
using the illustration in figure 14, it is possible to detect the WIMP
constituents of CDM. Only a brief explanation and result will be
mentioned by each of these phases as this begins to delve deeply into
particle physics.
Phase one:
Using CaF4 to
look for a 2β decay (to eliminate known leptons) an attempt was made to
determine signs of WIMP detection. The results were believed to be
successful, so phase two was designed an implemented.
Phase two:
Using 129Xe,
it was believed the sensitivity of this R&D phase identified three WIMP
particles believed to be photinos, higgsinos, and Majorana Neutrinos.
The success of this detection was lead to phase three – the actual
experiment.
Phase three:
LIBRA – Large sodium
Iodine Bulk for Rare processes.
NaI detectors built after
the two R&D phases ran for 4 years. The results of the experiment
determined there are particles that carry the qualifications to be
WIMP’s, but the results (available from the DAMA website) are confusing
at best.
Figure 15:
Reminiscent of the neutrino detectors, the DAMA experiment lies
deep underground to avoid interactions with any other particle
other than WIMP’s (this includes neutrinos, which are ruled out
by a specific reaction). |
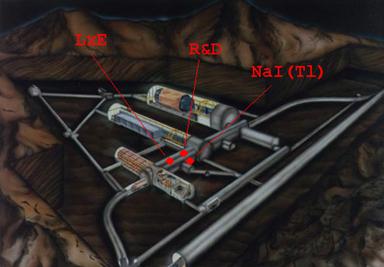 |
The DAMA project is
indeed a bold experiment, and certainly determined the existence of some
particles that match the requirements of WIMP’s, but to call them by
specific names is a bold assertion, especially without independent
collaboration. However, the results of the DAMA are already being
interpreted as solving the dark matter problems. The mirror symmetry
theory of particle physics states that every particle has a mirror
particle (not to be confused with matter and anti-matter). It is
asserted that mirror matter has been interpreted by the DAMA experiment
to be the sole constituent of CDM, solving for the large quantities
required to fit the model (Foot, 2004). Regardless, the results from
this experiment will need to be interpreted carefully and be confirmed
by other sources (as dictated by the scientific method).
WIMP detection using CaWO4
cryogenic detectors (Angloher et al., 2004):
One of the issues
acknowledged by attempting to detect WIMP’s is the ability to also
detect neutrinos. Regardless of what method used to detect the
particles, background suppression must also be designed into the
detector. The CREST-II project using CaWO4 cryogenic
detector, in its initial R&D and test run stage, promises to eliminate
false detection by implementing background suppression. This project is
sure to provide the much needed particle detection confirmation required
to interpret the data more effectively. The current status of this
project is still ongoing, but as of right now they have the ability to
constrain background noise introduced by weakly interactive particles
that are not the target WIMP’s.
Back to Top
| Back to
Cosmology
Self-Interacting Dark
Matter:
As mentioned several
times, the CDM model best fits our observable Universe except dwarf
galaxy evolution and halo density. Instead of abandoning the CDM theory
altogether, it is better to try and solve these issues within the model.
One answer is self-interacting dark matter. This is not a new particle
or a re-write of the theory; instead, the attempt is made to determine
if CDM particles interact with one another during galactic halo
evolution. This self-interacting (sometimes called collisional) dark
matter has been modeled to show that interaction with each other during
galactic halo formation has resolved some of the issues initially
generated by CDM simulations (Yoshida et al., 2000). Figure 16 shows an
example of Monte Carlo simulations on a local level (called high
resolution simulations by the Yoshida et al, 2000 paper).
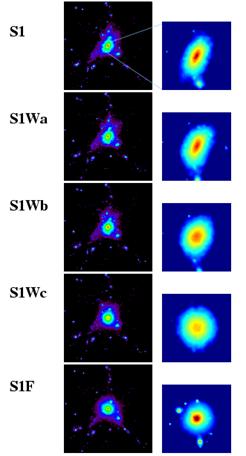 |
Figure 16:
An example of Self-Interacting Dark Matter formation of a dwarf
galaxy with variations of collisions between CDM particles:
S1:
no collision
S1Wa:
0.1 cm2/g
S1Wb:
1.0 cm2/g
S1Wc:
10.0 cm2/g
S1F:
Fluid Limit
(Yoshida et
al., 2000) |
What these results show
is the result of a gradually increasing interaction between particles.
The greater the interaction, the more spherical the model becomes. The
results of the Yoshida et al (2000) show that the S1Wb result best fits
observed and simulated densities of cores of these smaller simulated
structures.
Back to Top
| Back to
Cosmology
The Star Trek factor:
Dark Energy – which has
not been covered in this paper – begins to trail into the pure
theoretical with mathematics to match. However, one example of the Dark
Matter/ Dark Energy theory of interest is the role of the tachyon. You
may recall the mention of tachyons in various Star Trek: The Next
Generation episodes and I was actually a bit surprised that science is
lending them some credence. The importance of mentioning these particles
is their purpose to support the CDM model. While the mathematics are far
too advanced for the scope of this paper, it is generally thought that
the tachyon fields exists as sort of a super-fluid that provides the
energy comprising the Dark Energy theory, while at the same time
providing the support for the CDM particles (Herrera, Pavon and Zimdahl,
2004). If that seems a bit confusing (and I’m sure it does), the
importance of this field is to provide support for the structure of the
CDM filaments as the Universe expands.
Back to Top
| Back to
Cosmology
Summary and Final
Thoughts:
The Cold Dark Matter
model is the current accepted theory of dark matter. While the two main
issues are problems with galactic halo formation and the dwarf galaxy
problem, this apparently can be addressed by looking carefully at how we
model the simulations in a supercomputer (instead of abandoning the
model itself). At the same time observational evidence of WIMP particle
detection, constraints of the age of the Universe by the WMAP, and
examination of radio jets of large galaxy support the CDM theory while
ruling out the WDM and HDM models. The purpose of Tachyonic and
Self-Interacting Dark Matter is also used to help solve the issues with
the CDM model while also addresses the Dark Energy problem as well (not
covered here). Dark matter most certainly exists and while we currently
have no idea what dark matter is, we are rapidly closing in on finally
detecting dark matter particles directly. While there are sharply
divided camps in the theory of dark matter, more and more theorists are
acknowledging the CDM model and are focusing their energy into helping
smooth out the difficulties. The attempts made by the DAMA experiment
and the CaWO4 cryogenic detectors are important to mention,
but until their results are independently confirmed by other detectors
we should hold off on opening the Champagne bottles. One thing is
certain: we are closer to identifying the constituents of CDM and the
mystery of dark matter is certain to be solved in the very near future.
Back to Top
| Back to
Cosmology
Image Credits:
Figure 1:
http://www.owlnet.rice.edu/~spac250/elio/spac.html
Figure 2:
http://www.owlnet.rice.edu/~spac250/elio/spac.html
Figure 3:
http://einstein.stanford.edu/content/education/EducatorsGuide/Page7.html
Figure 4:
http://www.star.ucl.ac.uk/~idh/STROBEL/evolutn/evolutnc.htm
Figure 5:
http://www.star.ucl.ac.uk/~idh/STROBEL/evolutn/evolutnc.htm
Figure 6:
http://www.star.ucl.ac.uk/~idh/STROBEL/evolutn/evolutnc.htm
Figure 7:
http://www.star.ucl.ac.uk/~idh/STROBEL/evolutn/evolutnc.htm
Figure 8:
http://antwrp.gsfc.nasa.gov/apod/ap011007.html
Figure 9:
http://perry.sonoma.edu/journeys/dark_matter/pages/baryonic.html
Figure 10:
http://astron.berkeley.edu/~mwhite/modelcmp.html
Figure 11:
http://astron.berkeley.edu/~mwhite/modelcmp.html
Figure 12:
http://cfa-www.harvard.edu/cpac/Reion/stars.html
Figure 13:
http://cfa-www.harvard.edu/cpac/Reion/stars.html
Figure 14:
http://www.lngs.infn.it/lngs/htexts/dama/welcome.html
Figure 15:
http://www.lngs.infn.it/lngs/htexts/dama/welcome.html
Figure 16:
http://www.mpa-garching.mpg.de/~naoki/SIDM.html
Back to Top
| Back to
Cosmology
Web Sites:
DAMA Experiment:
http://www.lngs.infn.it/lngs/htexts/dama/welcome.html
Simulations of Local
Universe:
http://www.mpa-garching.mpg.de/HIGHLIGHT/2001/highlight0107_e.html
LSST Observatory:
http://www.lsst.org/Science/darkmatter2.shtml
Galaxy Rotation Curve:
http://www.astro.utu.fi/~cflynn/rotcve.f1.html
Back to Top
| Back to
Cosmology
References:
Angloher, G, et al.
“Limits on WIMP Dark Matter using Scintillating CaWO4 Cryogenic
Detectors with Active Background Suppression.” ApJ, in press,
astro-ph/0408006v2.
Bode, Paul, Jeremiah
Ostriker and Neil Turok. “Halo Formation in Warm Dark Matter Models.”
The Astrophysical Journal, 556:93-107, 20 July 20, 2001.
Brook, Chris, Daisuke
Kawata, Brad Gibson and Ken Freeman. “The Emergence of the Thick Disk in
a Cold Dark Matter Universe.” The Astrophysical Journal, 612:894-899,
September 10, 2004.
Flynn, Chris, Andrew
Gould and John Bahcall. “Hubble Deep Field Constraint on Baryonic Dark
Matter.” The Astrophysical Journal, 466:L55-L58, August 1, 1996.
Gibson, Brad and Chris
Flynn. “White Dwarfs and Dark Matter.” Science Magazine, Volume 292,
June 22, 2001.
Gondolo, Paolo.
“Non-Baryonic Dark Matter.” ApJ, in press, astro-ph/0403064v1.
Herrera, Ramon, Diego
Pavon, and Winfried Zimdahl. “Exact Solutions for the Interacting
Tachyonic-Dark Matter System.” ApJ, in press, astro-ph/0404086v1.
Kaplinghat, Manoj and
Michael Turner. “How Cold Dark Matter Theory Explains Milgrom’s Law.”
The Astrophysical Journal, 569:L19-L22, April 22, 2002.
Ma, Chung-Pei and Edmund
Bertschinger. “A Cosmological Kinetic Theory for the Evolution of Cold
Dark Matter Halos with Substructure: Quasi-Linear Theory.” The
Astrophysical Journal, 612:28-49, September 1, 2004.
Metcalf, Benton. “The
Detection of Pure Dark Matter Objects with Bent Multiply Imaged Radio
Jets.” The Astrophysical Journal, 580:696-704, December 1, 2002.
Milgrom, Mordehai. “Do
Modified Newtonian Dynamics Follow from the Cold Dark Matter Paradigm?”
The Astrophysical Journal, 571:L81-L83, June 1, 2002.
Reid, Neill, Kailash Sahu
and Suzanne Hawley. “High-Velocity White Dwarfs: Thick Disk, not Dark
Matter.” The Astrophysical Journal, 559:942-947, October 1, 2001.
Root, R. “Exploring the
Mirror Matter Interpretation of the DAMA experiment: Has the Dark Matter
Problem Been Solved?” ApJ, in press, astro-ph/0403043.
Silk, Joseph. A Short
History of the Universe. Scientific American Library, New York.
1999.
Yoshida, Naoki, Aaron
Sokasian, Lars Hernquist and Volker Springel. “Early Structure Formation
and Reionization in a Warm Dark Matter Cosmology.” The Astrophysical
Journal, 591:L1-L4, July 1, 2003.
Yoshida, Naoki, Volker
Springel, Simon White and Giuseppe Tormen. “Weakly Self-Interacting Dark
Matter and the Structure of Dark Halos.” The Astrophysical Journal,
544:L87-L90, December 1, 2000.
Back to Top |
Back to Cosmology |